Introduction
Carbon capture and storage (CCS) involves capturing CO2 from point-source surface facilities and injecting it into subsurface geological formations, particularly depleted oil and gas reservoirs, unmineable coal seams or deep saline aquifers. In 2008 the government of Alberta committed CDN$2B to accelerate CCS in the province, with the goal of having several > 1Mt/yr CCS projects operating by 2015. Current targets are to have 50 million tonnes of CO2 stored annually by 2020 and 139 million tonnes annually by 2050. Of paramount importance to CCS is the ability to track CO2 plume movement, if possible, and to assess whether the CO2 flow is consistent with model predictions, and to optimise safeguards for possible early leak detection. These goals are vital for public acceptance of CCS, particularly during early projects as the technology becomes implemented at commercial scale. Comprehensive yet affordable monitoring protocols thus need to be established. A wide range of technologies has been developed for monitoring CO2 injection and storage, including surface and subsurface measurements and surveys, but continued research and development is required for next generation technologies that will enable effective monitoring programs to be implemented. This represents both opportunities and challenges for geophysics. These will be discussed in this presentation.
Background
CCS is being undertaken in a number of countries, and current best practices have been documented for sites in Europe (Chadwick, 2008), Australia (Kaldi and Gibson-Poole, 2008), and North America (NETL, 2009). At Sleipner in Norway, one of the longest established CCS sites, the measurement, monitoring and verification (MMV) plan implemented is based on time-lapse seismic surveys which have been very successful in mapping the CO2 plume. The storage reservoir at Sleipner (Utsira Sand) is thick (~ 200 m), with porosity of up to 35% (Kaldi and Gibson-Poole, 2008), so that the geophysical signature of the CO2 plume is unequivocal. However, the properties of the Utsira Sand are not typical of storage formations at other CCS sites and the monitoring results from Sleipner should not necessarily be used to illustrate what to expect from site characterisation and monitoring programs in Canada. This is particularly true in the Western Canada Sedimentary Basin (WCSB) where strata are consolidated or tightly cemented and storage reservoirs are generally thin (< 100 m thick) and may have low porosity and permeability; e.g. Pembina Cardium Field (Lawton, 2009). Also in Canada, the well-known Weyburn Project in Saskatchewan illustrates subtle traveltime and amplitude anomalies in time-lapse seismic data used to monitor a CO2 enhanced oil recovery project hosted in relatively thin dolomite reservoir (White et al., 2004).
CO2 storage in the WCSB
Geological storage of CO2 in the WCSB generally falls into two families. The first is in depleted oil and gas reservoirs where CO2 may be used for enhanced oil and gas recovery or for outright storage once production has declined (Figure 1a). The advantages of this scenario are that the reservoir geometry and properties are well known, there is a physical or well-understood stratigraphic trapping mechanism, the cap rock integrity is established and fluid flow within the reservoir has often been historymatched at production wells. On the other hand, there are usually a significant number of producing or abandoned wells that penetrate the reservoir and some of these may constitute leakage paths to shallow aquifers or to the ground surface.
The second storage family is in deep (> 1 km) saline aquifers (Figure 1b), generally hosted in Paleozoic carbonates in the WCSB. The advantages of saline aquifer storage is the available pore space volume is significantly greater than that available in depleted hydrocarbon reservoirs (Bachu and Gunter, 1999), and the number of well penetrations is small thus reducing possible leakage paths through wells. In contrast, the disadvantages are that the reservoir may not be confined, the integrity of the cap rock may be poorly known and there is no history match to understand fluid flow in the reservoir. This requires that a comprehensive site assessment and reservoir characterization be undertaken before large-scale CO2 injection is started.
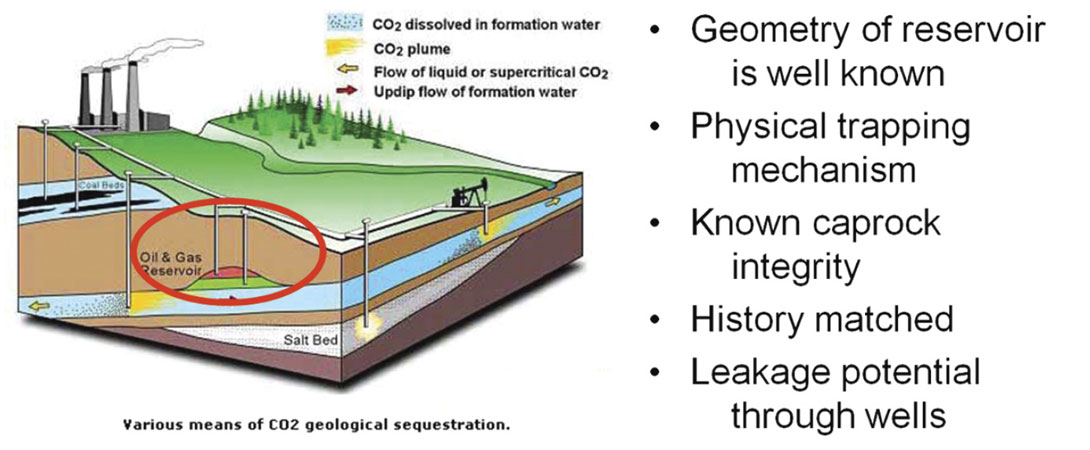
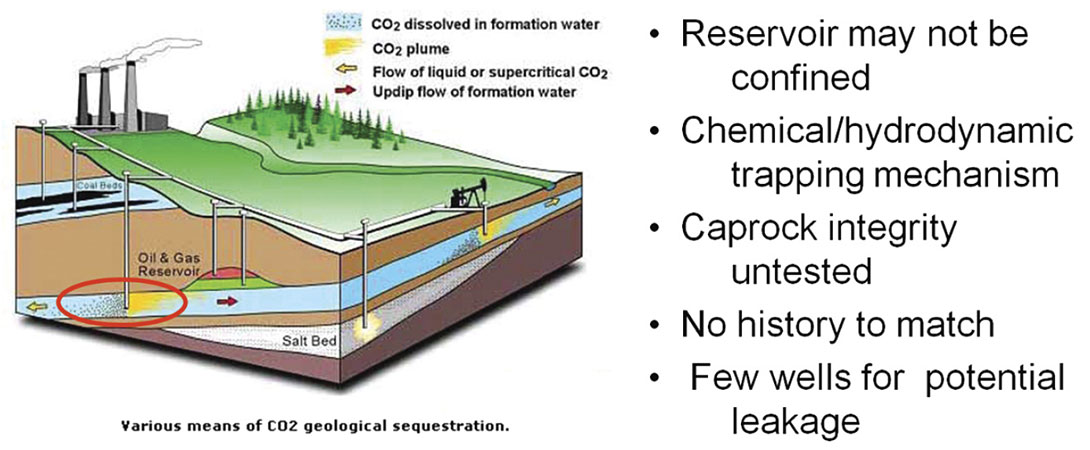
Properties of CO2
In order to maximize storage capacity, CO2 is typically injected into a storage formation in a supercritical state, at which it has interesting properties of both the liquid and gas phases. Figure 2 shows a phase diagram of CO2, with the critical point at 31.1 degrees C and 7.3 MPa pressure, typically reached in the Earth at depths greater than about 800 m. At 35 degrees C and 10 MPa, CO2 has a density of 650 kg/m3, a Pwave velocity of 332 m/s and a bulk modulus of 72 MPa. In comparison, at similar PT conditions, brine with 50,000 ppm NaCl has a density of 1032 kg/m3, a P-wave velocity of 1585 m/s and a bulk modulus of 2592 MPa. Typically the change in seismic properties of a rock unit with partial or full replacement of brine with supercritical CO2 is calculated using the Gassmann equations (Gassmann, 1951), or more recently, Biot- Gassmann approaches. The changes in seismic properties are manifested by changes in rock moduli and a resulting decrease in P-wave velocity which in observed in traveltime delays through the CO2 plume and changes in reflectivity (impedance) of the horizon (e.g. Sodagar and Lawton, 2009). In these computations, the shear modulus is assumed to be unaffected by fluid substitution so the S-wave velocity is predicted to increase slightly with increasing CO2 saturation due to the small decrease in bulk density. In the WCSB changes in interval P-wave velocities are likely to be less than 10% and a time-lapse seismic difference will be difficult to detect from surface seismic data for CO2 injected into thin reservoirs. If the saturation is uniform, then the change in velocity is non-linear with CO2 saturation and little change is observed between about 40% and 100% CO2 saturation. Thus, any determination of the CO2 volume stored from seismic inversion is at best an estimate.
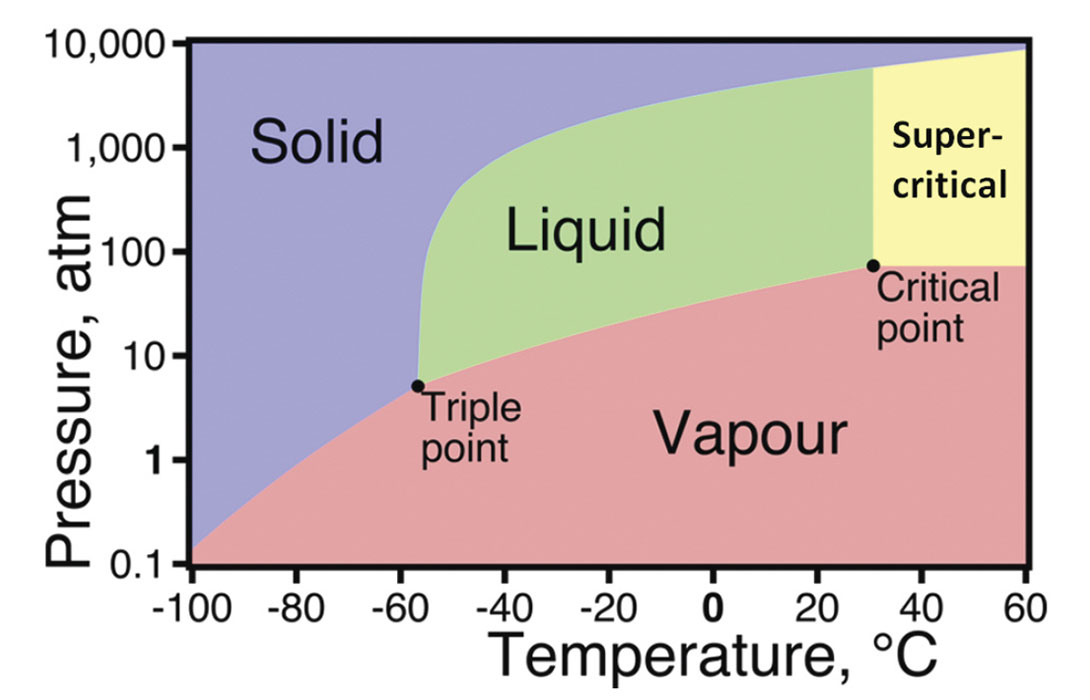
Site characterization example
For CO2 storage in saline aquifers, regional information about the aquifer is required. For example, the University of Calgary has recently completed a regional study to assess CO2 storage potential in the Nisku Formation over an area of 5000 km<sup>2</sup> southwest of Edmonton. Seismic characterization of the Nisku Formation in the Wabamun Area CO2 Sequestration Project (WASP) was based on analyzing and interpreting poststack seismic datasets comprising more than two hundred 2D seismic lines and seven 3D volumes (Alshuhail et al., 2009a). The study area is surrounded by two major hydrocarbon resources in Alberta; the Leduc reef play (east) and the Moon Lake reef play (northwest). Thus, part of the study area was mapped using vintage surface seismic data that had been acquired as part of hydrocarbon exploration in the area. The 2D and 3D seismic datasets have different acquisition and processing specifications and were acquired over many years prior to this project being undertaken. Prior to interpretation, inversion and attribute analysis, two primary steps were undertaken: data calibration and amplitude normalization. These steps were necessary to account for the vintage and datum differences within the data. Subsequent inversion of the seismic data revealed contrasts in acoustic impedance caused by lateral changes in lithology and/or porosity of the Nisku Formation (Figure 3). The analysis revealed favourable low-impedance, high-porosity locations that could be potential injection sites.
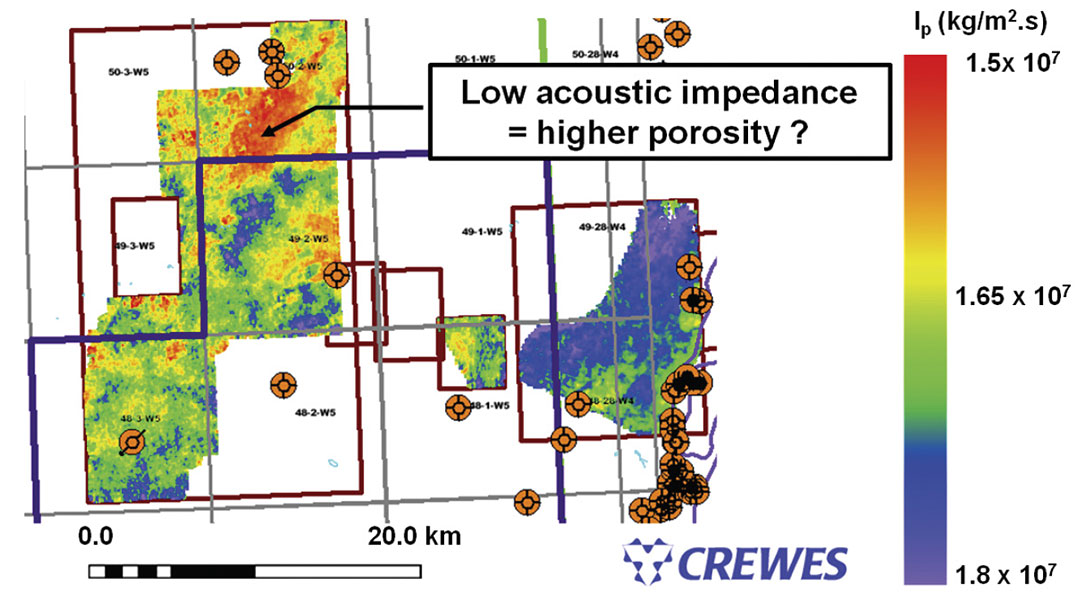
Monitoring example
We have undertaken seismic monitoring of CO2 injection at two sites in Alberta, with other projects anticipated to be starting in 2010-2011. At Alder Flats, a high-resolution 3D seismic program was recorded at a site where enhanced coalbed methane production and carbon sequestration were being tested (McCrank and Lawton, 2009). The surface seismic data were collected several months after 180 t of CO2 had been injected in the Ardley coals at a depth of 420 m below surface. Poststack inversion of the data showed a low acoustic impedance anomaly up-dip and along strike of the preferential fluid pathway in the target coal zone, as illustrated in Figure 4. The size and location of the anomaly matches the expected imprint of the injected CO2 but the magnitude of the apparent acoustic impedance reduction cannot be explained by the Gassmann fluid substitution model, suggesting that the elastic moduli of the coal rock frame has been reduced by exposure to the CO2.
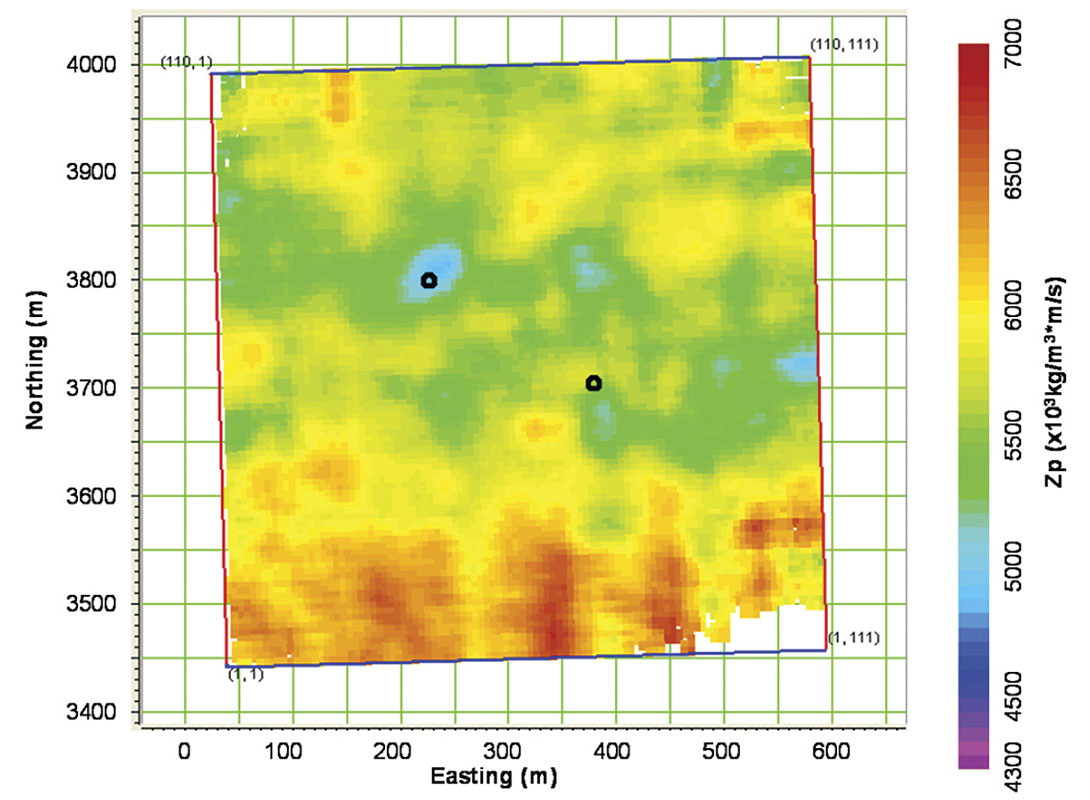
In the Pembina Field, sparse baseline and two repeat seismic surveys were recorded over a period of two years to monitor a CO2 enhanced oil recovery project in the Cardium Formation (Lawton, 2009; Alshuhail et al., 2009b). Timelapse analysis of the surface seismic dataset showed no significant anomaly that could be attributed to the injected supercritical CO2 between Phase I (March 2005) and Phase III (March 2007) of the project (Figure 5).
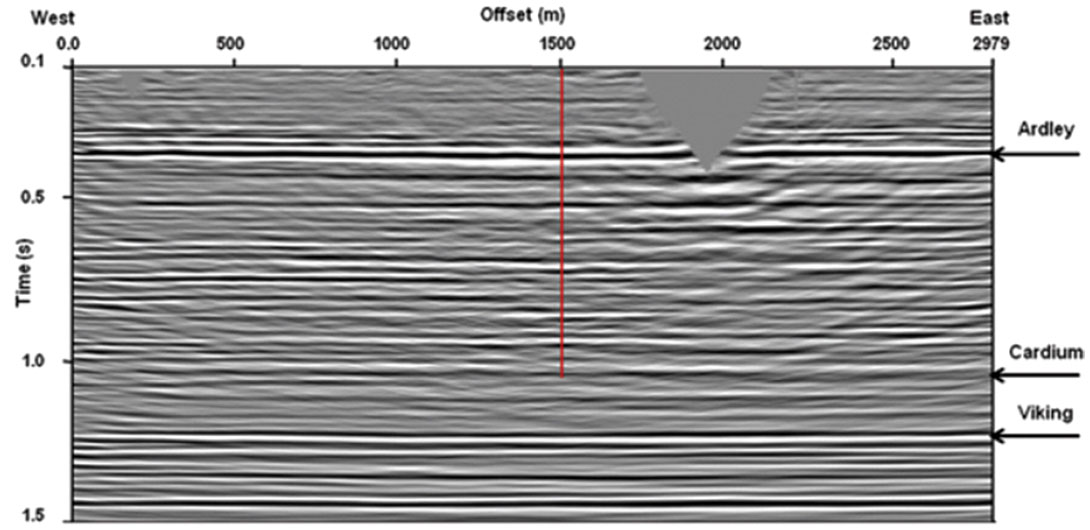
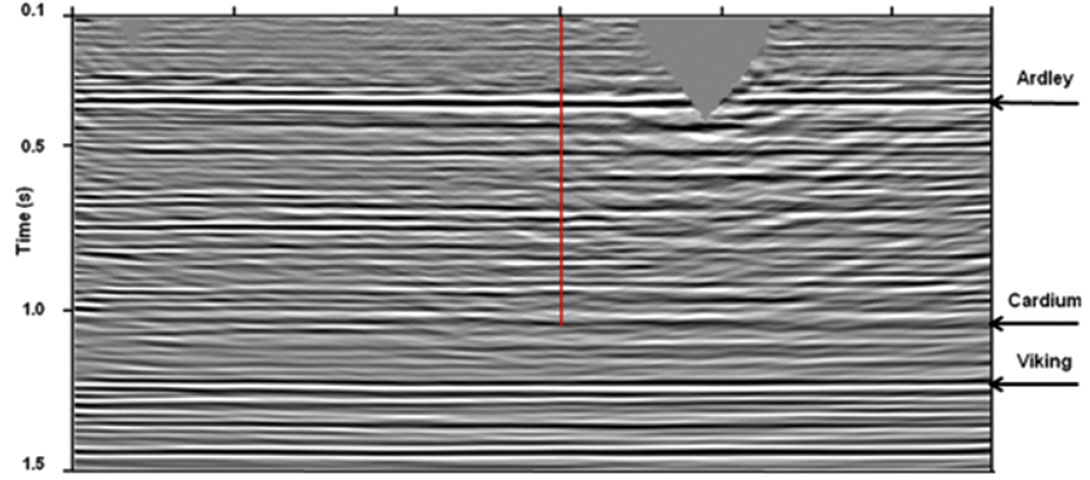
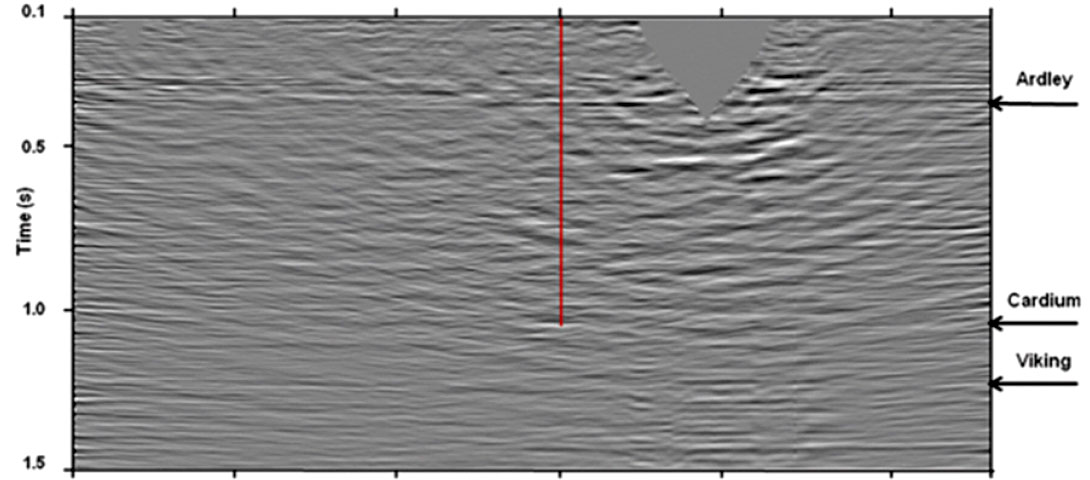
However, a fixed-array walkaway vertical seismic profile (VSP) dataset exhibited small amplitude variations that may be associated with the CO2 plume (Figure 6). The time-lapse analysis was based on the observation of amplitude and traveltime variations after the injection of approximately 40,000 t of CO2 into the 1650 m deep, 20 m thick Cardium Formation over the period of two years. The analysis suggests that it will be hard to detect the CO2 plume with surface seismic data since it is interpreted to be contained in thin layers of relatively permeable sandstone members of the Cardium Formation. However, walkaway VSP surveys show more promise. The monitoring program indicated that no leakage of CO2 had occurred into shallower formations where the CO2 would be in a free gas phase.
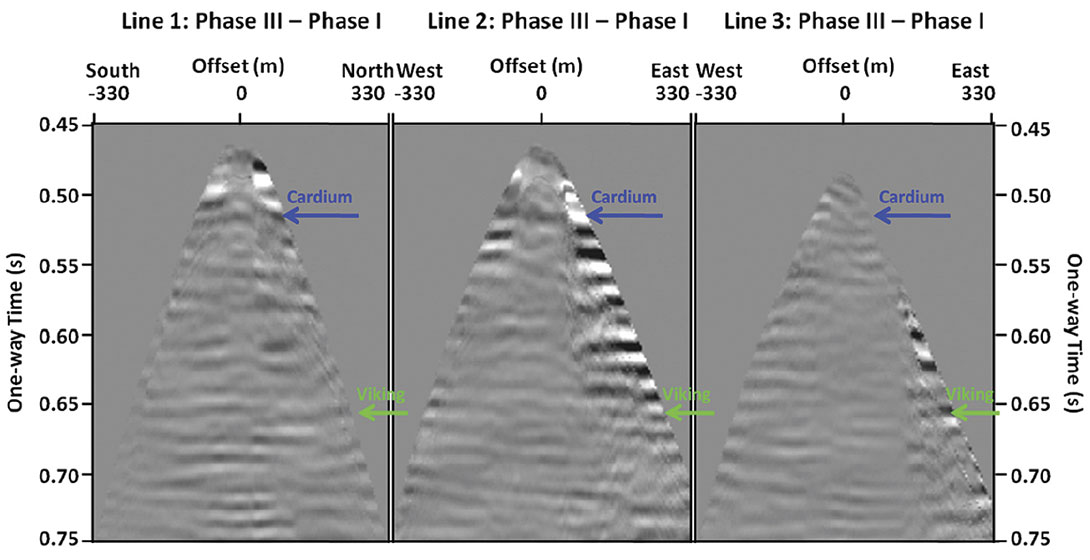
Discussion
Monitoring of CO2 storage in the WCSB will be challenging but represents a significant opportunity to refine time-lapse seismology. The principal applied technology for monitoring CO2 storage remotely is seismic imaging but necessary supporting technologies include well logging, gravity surveys, and tiltmeters and interferometric synthetic aperture radar (InSAR) for surface deformation measurements. Integration of geophysical, geochemical and geomechanical datasets will also need to be undertaken. However, seismic imaging holds a principally important role for monitoring because no other method can match its volumetric coverage and resolution, but major issues relate to poorly constrained and highly non-linear seismic response-gas saturation relationships and the poor repeatability of seismic surveys. Moreover, the inversion of seismic data for physical subsurface properties is complex and essential constraints are provided by the supporting technologies, together with petrophysics and rock property measurements. For CCS in general, potential challenges exist in the WCSB for CO2 capacity, injectivity, pressure management and resource interference. To investigate some of these, the University of Calgary is spearheading the development of a CCS-MMV field research and training centre near Calgary.
Acknowledgements
Much of the research contributing to this presentation was undertaken by graduate students Marcia Coueslan, Fuju Chen, Jason McCrank, Abdullah Alshuhail and Taher Sodagar. The Nisku characterization project was funded by the WASP consortium at the University of Calgary and the Natural Sciences and Engineering Research Council of Canada (NSERC); support for the Pembina-Cardium CO2 monitoring project was provided through the Alberta Energy Research Institute (AERI) of the Province of Alberta, Western Economic Diversification (WED), Natural Resources Canada (NRCan), grants from NSERC, Penn West Energy Trust; the Ardley coals project was supported by the sponsors of the CSEMP Consortium, NRCan, AERI and NSERC. We acknowledge the CREWES project, Hampson- Russell Software, Landmark Graphics and Fugro-Jason for assistance in for providing access to software.
Join the Conversation
Interested in starting, or contributing to a conversation about an article or issue of the RECORDER? Join our CSEG LinkedIn Group.
Share This Article