Exploration in frontier basins is challenging; subtle targets, large seismic datasets, sparse well penetrations, and many unknowns. There is an obvious requirement for interpretations to be robust, but also delivered on a commercial timeframe.
In order to make robust and informed interpretations of seismic amplitudes, we need to understand the geological controls on the elastic properties of each seismically distinct rock type that may be present within the basin; we also need to understand if some rocks can’t be imaged. The geological properties that we are interested in, and those that control elastic properties, include the rock mineralogy, porosity, fluid saturation, depth of burial, and compaction state, as well as pore pressure and stress. To make this connection we need to turn to rock physics.
In order to establish links between the geological properties of interest and the seismic responses observed away from well control, we often turn to well data. In frontier basins, well control is often limited, and as a consequence, understanding the geological signal behind the seismic observations can be difficult.
In this paper, discussion of the key considerations that should be made when developing a predictive rock physics framework is provided. This is illustrated by reference to an example from the Canadian Atlantic margin (see Huntbatch, et al 2016). Detailed information on this regional study can be found on the Nalcor Energy website (https://nalcorenergy.com).
A facies-dependent rock physics framework
The seismic responds to contrasts in the elastic properties across interfaces between different rock types. We need to understand the geological controls on those elastic properties; this is where rock physics comes in. Rock physics is the link between geological properties, processes, and the elastic properties of the rocks.
For the framework to be useful for seismic interpretation, it needs to capture the rock physics trends per facies, and also be capable of perturbing those trends based on the geological variables of interest. We can then use the framework to generate multiple geometric models for a given prospect location based on different geological scenarios. The scenarios selected should be informed by basin modelling and geological understanding.
Facies
We are interested in seismically distinct rock units, or elastic facies (here we will use the term facies, but this should not be confused with other definitions of the word). These are rock units with seismically distinct elastic properties. How distinct, or otherwise, the elastic properties of each facies are is dependent on the same geological properties and processes that we aim to capture in the rock physics framework. With this in mind, another key aim of the framework is understanding the spread of elastic properties for each facies, the uniqueness of the responses, and ultimately the geological and data uncertainties in interpreting the seismic response.
The first step is therefore to identify what facies might be present in the basin of interest. This is done by looking at regional well data, analogue data, publications, and basin modelling. Where well data is sparse, analogues become increasingly important.
Geological processes and rock physics
So what geological properties do we need to consider when building the predictive framework? The answer to this will depend on the basin and the facies types involved, and also whether the prospective location is shallower or deeper than the available well control. In general the following geological parameters should be considered.
- Basin and burial history
- Fluid saturation in porous zones
- Mineralogy
- Elastic anisotropy
- Pore pressure and stress
How the impact of these parameters on the elastic response is captured again depends on the facies types that are relevant, and a mix of empirical, theoretical and heuristic models can be combined to develop the framework. A thorough review of the various rock physics concepts and models is provided by Mavko et al., 2009.
In the example from the Canadian Atlantic margin, empirical trends were developed for the non-reservoir zones, a heuristic contact-type rock physics model was calibrated for the reservoir sandstone data (see Avseth, et al, 2005), and Gassmann’s relations (Gassmann, 1951) were used to predict the effect of pore fluids in the sands. In addition, empirical trends between elastic properties and Vertical Effective Stress (VES) were developed to investigate the impact of pore pressure in both the reservoir and non-reservoir intervals.
This type of work depends on the universality of rock physics trends, which means that individual geological processes have predictable effects on the elastic properties of the rocks; see Selnes et al., 2015, for an expansion on this concept. Focus on the effect of compaction, anisotropy and stress will be made here.
Compaction and porosity
When looking at velocity or porosity data from basins worldwide, a consistent pattern emerges (see Bjørlykke, 2014). In the shallow section (above the ~70°C isotherm) mechanical compaction dominates. In shales this takes the form of the collapse and reorientation of platy clay minerals; the result is a rapid increase in velocity and decrease in porosity in the first few kilometres of burial depth. In sandstones the porosity loss and velocity increase is much milder; this is related to sorting and crushing of grains. In the deeper sections (below the ~70°C isotherm), the compaction regime changes to one dominated by chemical processes such as smectite-illite transformation in shale and quartz overgrowth in the sandstones. Sandstone porosity depth trends from the Atlantic Canada study are shown in Figure 1. This trend was defined with reference to analogue data from Mid-Norway; the use of analogues is discussed later. The error bars on this trend capture the spread of porosity values per depth, and can be fed into the rock physics modelling phase to understand uncertainty in elastic responses.
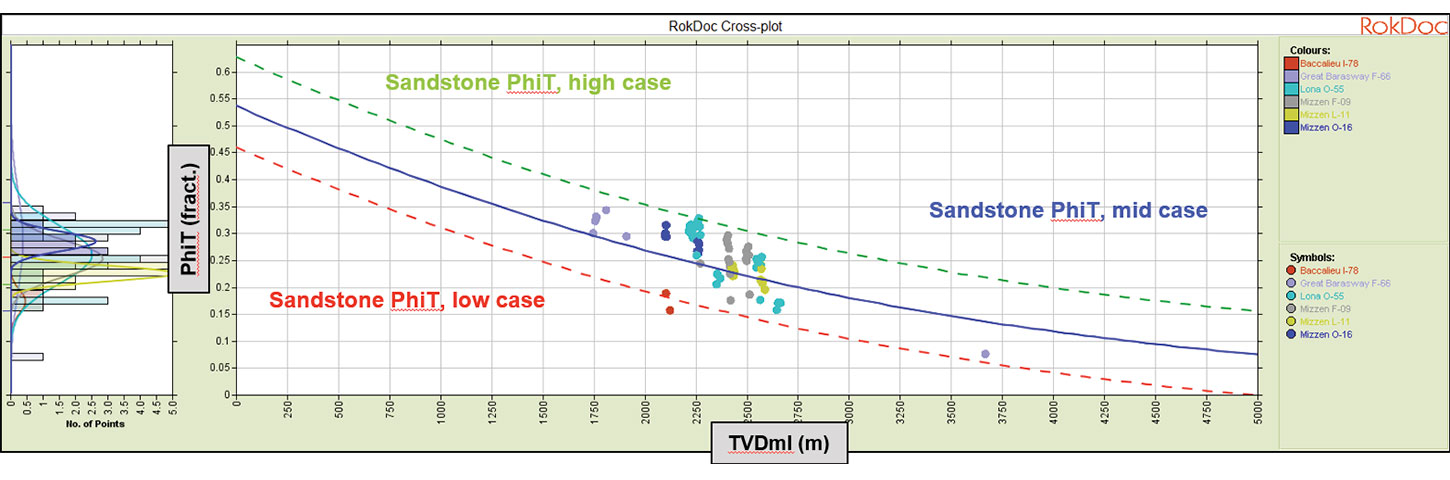
The impact on rock elastic properties of these different compaction regimes has been noted in basins worldwide (see Huntbatch, et al., 2016). The sandstone p-wave velocity versus porosity data from the 2016 Atlantic Canada study is shown in Figure 2. There is a distinct shift in trend from the shallow to the deep data, and again this pattern of responses has been noted in many studies (e.g. Dvorkin and Nur, 1996). Capturing this effect can be a key part of any predictive rock physics framework, and this can be achieved via the use of calibrated rock physics models such as that shown in Figure 2. The p-wave velocities as a function of burial depth predicted by this model are plotted in Figure 3. Here a trend is calculated for each compaction state, from mechanically compacted to high levels of grain contact cement.
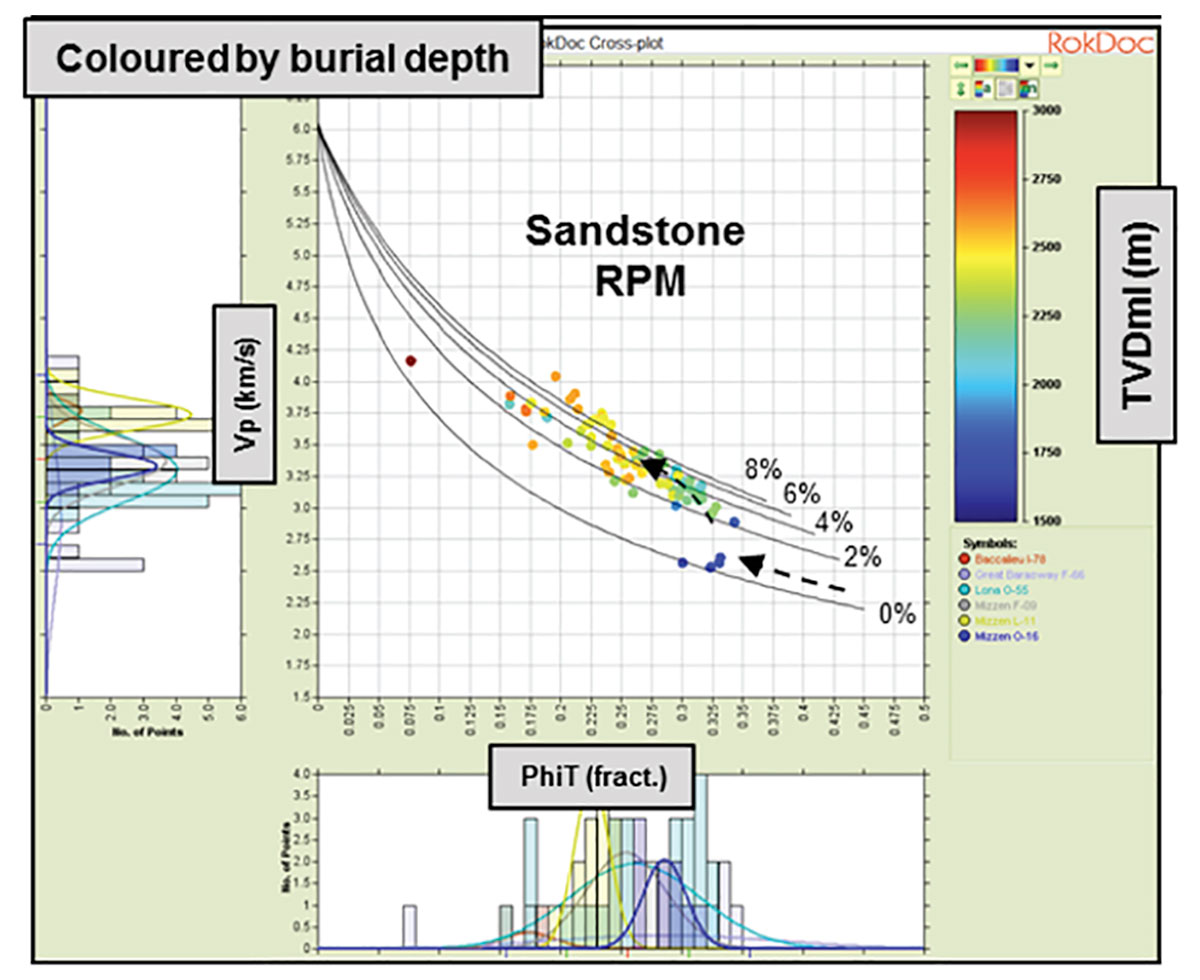
Clearly it is also important to consider and incorporate the burial and exhumation history of the basin. Are the rocks of interest at their maximum burial depth? Is under or over compaction at play?
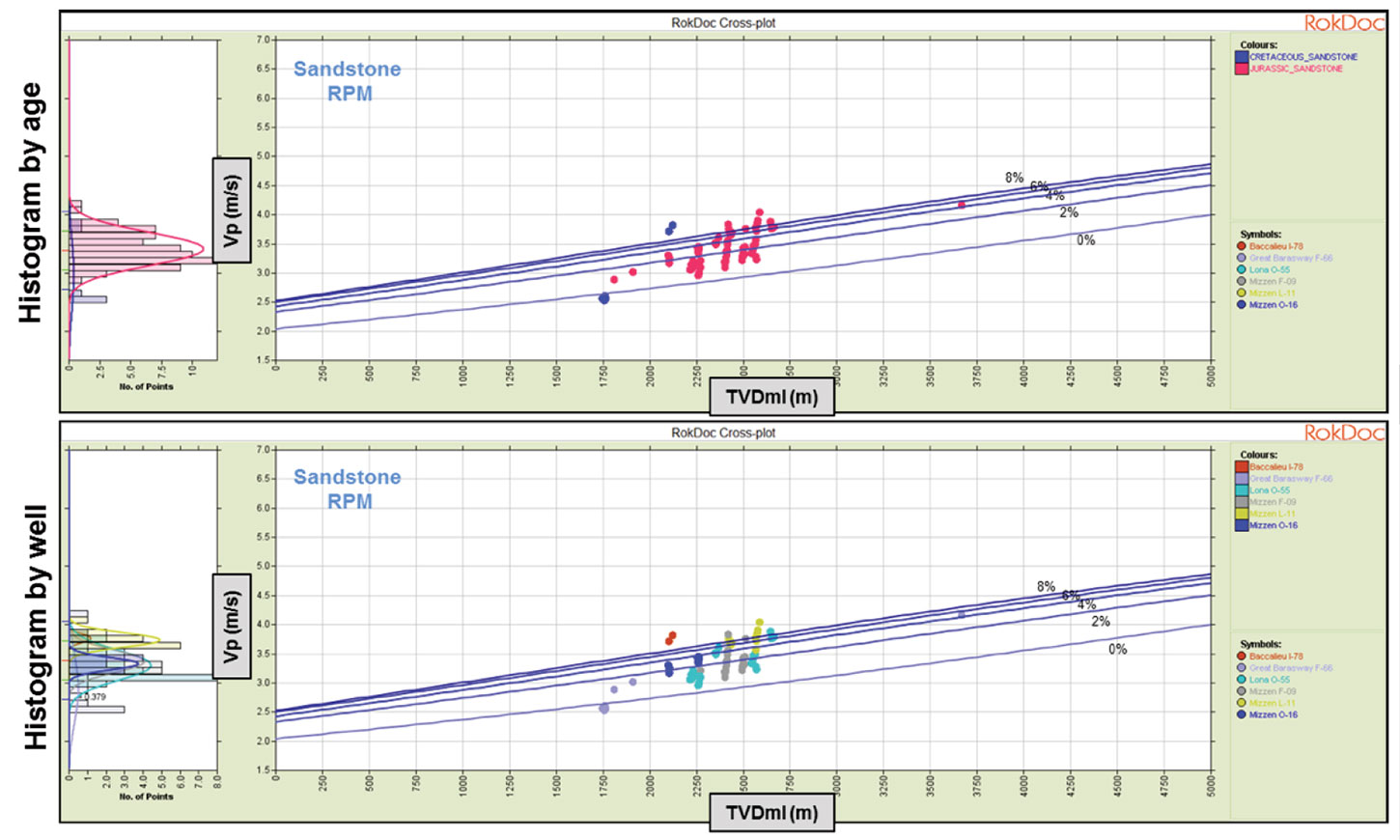
Elastic anisotropy
Anisotropy is ubiquitous in the subsurface. In most cases it is ignored, with the assumption, or hope, being that the effect of anisotropy is small. This is not always the case, and anisotropy can have a strong effect on the observed seismic AVO response. In particular anisotropy related to shale layering fabric (Vertical Transverse Isotropy – VTI) in shales can lead to strong effects on AVO gradient. Calibrating anisotropy models in frontier exploration settings can be difficult given the lack of data; however, empirical approaches such as that of Ryan-Grigor (1998) can be used to get a first estimate of the anisotropic parameters based on the Vp/Vs ratio of the rocks. Once a sensible set of anisotropic model parameters have been defined, an anisotropic reflectivity equation (e.g. Ruger, 1997) can be used to generate anisotropic synthetic gathers. In any case, an anisotropic shale scenario should be included when investigating the seismic response at any prospect. The normal sanity checks should be applied when considering the effects of anisotropy on seismic amplitude: amplitude geometry, reflector terminations, amplitude conformance to structure, etc.
Pore pressure and stress
Another important geological control on the elastic properties of the rocks is the effective stress. This is controlled by the nature of the confining stress, which is dependent on the overburden thickness, the tectonic regime in the basin, and the pressure within the rock pore space. Generally at high pore pressure and low effective stress, the rocks are relatively soft. At low pore pressure and high effective stress, the rocks tend to be relatively stiff. Capturing this effect and including it within the framework is key where high pore pressures are expected. Elevated pore pressure can be one cause of under-compaction, where soft rocks are encountered in the deep section.
Regional Knowledge and Analogues
A note should also be made regarding analogue data. Inclusion of all relevant regional (or supraregional) knowledge is key to the successful construction of a predictive rock physics framework. This data can take the form of offset wells within the basin, publications and reports, and also data from analogous basins. An example is shown in Figure 4, where data from Mid-Norway is used to reinforce the definition of a porosity-burial depth trend for use in the Orphan Basin and Flemish Pass, Atlantic Canada (see Huntbatch, et al., 2016). This allowed an increase in confidence when predicting porosity at a given prospect location in these basins, and allowed for a more robust input into the calibrated rock physics model used to predict the elastic properties of the sandstones.
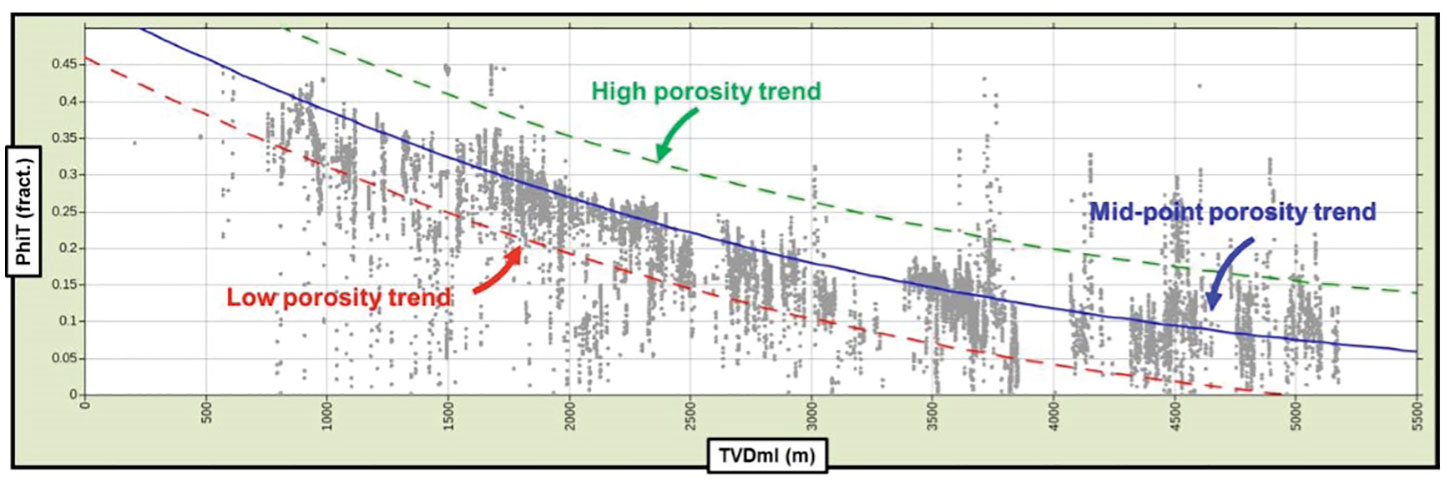
Applications and examples
Once the framework has been constructed, it should be validated at the available well penetrations to give confidence in its predictive power. The outputs from such a study have broad uses, from AVO modelling to seismic inversion and beyond.
AVO models
The primary use of the framework in frontier exploration settings will be the prediction of elastic properties at a prospect depth for each facies, and the generation of multiple reflectivity model scenarios that can be used to inform the interpretation of the seismic response. Once elastic properties can be predicted per facies, any number of different geometric models can be generated of varying complexity as required. The spread of responses captured within the framework can also be used to understand the geological uncertainty in interpretation.
Seismic inversion
Other applications of the trends that are generated by the kind of framework described here can include use in seismic inversion. Per-facies trends can be used to provide the low frequency content for seismic inversion, with the framework allowing the extrapolation of these trends away from well control. A good example of this is shown by Somoza et al., 2015.
Conclusions
A robust per-facies predictive rock physics framework is a must if informed interpretations of seismic responses are to be made. In order to generate such a framework, we must understand the connection between the relevant geological properties and processes and the elastic properties of each facies. Key controls include burial history, compaction state, pore pressure, and elastic anisotropy. In an exploration setting, many of these parameters can only be captured with the inclusion of regional knowledge and data from analogous basins.
It is important to include information from multiple disciplines in order to correctly define the framework; these disciplines include basin modelling and pore pressure.
Once this framework is in place, we can generate multiple scenarios by perturbing the geological parameters of interest; these scenarios can then be used to inform seismic interpretation. Finally it should be noted that the type of work described here forms one element of the de-risking workflow and should be considered in conjunction with other geoscience disciplines when evaluating a prospect.
Acknowledgements
The author would like to thank Alsing Selnes, Neil Whitfield and Nalcor Energy for the preliminary work done in the regional study, Atlantic Canada (https://nalcorenergy.com/).
Join the Conversation
Interested in starting, or contributing to a conversation about an article or issue of the RECORDER? Join our CSEG LinkedIn Group.
Share This Article