Summary
Oil production from low-permeability strata in the Western Canada Sedimentary Basin (WCSB) is continuously increasing, rivaling the production potential from conventional targets in the same areas. This increase in productivity is related to important advances in drilling and completion technology that are driven, in particular, by improvements in multi-fractured horizontal well technology. In spite of these advances, finding the best combination of reservoir properties within a given stratum remains a challenge among geoscientists and reservoir engineers. As a result reservoir characterization remains an important step in the low-permeability reservoir development process. We provide below a summary of several advanced characterization techniques, previously applied to the study of other types of unconventional reservoirs, which we have adapted and are using to evaluate the spectrum of properties observed in tight-oil or halo-oil reservoirs.
Introduction
Unconventional light oil accumulations are quickly reshaping the oil production map for several traditional plays across North America. These changes have been undoubtedly associated with the continuous improvement of drilling and completion strategies, along with sustained favorable market conditions for this commodity. Within the WCSB, examples of this evolving trend include the Cardium, 2ndWhite Speckled Shale, Viking, Montney, and Bakken/Exshaw formations. These unconventional light oil plays can be classified as “tight-oil”, “halo-oil”, and “shaleoil”, depending on the characteristics of the dominant reservoir/fluid system (Clarkson et al, 2011). This report focuses primarily on the characterization of halooil plays, in which the reservoir is not self-sourced, and where the matrix permeability is often less than, but can exceed, 0.1 md (e.g., as is the case in low-permeability areas of the conventional Cardium and Viking pools in Alberta).
The major breakthrough leading to the successful development of low-permeability hydrocarbon reservoirs was the introduction of horizontal wells completed with multiple hydraulic fracturing stages. This practice effectively, maximizes the wellbore-reservoir contact area in these low-permeability rocks. In spite of these technological advances, unconventional light oil reservoirs exhibit a wide variety of geological characteristics that make difficult the application of a global or allencompassing completion/development recipe. For instance, rock heterogeneity observed in key properties at multiple reservoir scales often requires the investigation of different scenarios, and the selective application of mixed completion methodologies to guarantee the success of the project. Consequently, a better understanding of these multi-scale controls is required to improve reservoir performance. The identification and mapping of properties along with other reservoir quality indicators is of primary importance for the assessment and successful exploitation of these resources.
Characterization Methods
A wide variety of characterization tools and methods are usually required to properly portray the complexity found in an average hydrocarbon reservoir (Figure 1). The major principles behind these tools depend upon the scale, resolution, and nature of the measurement itself. Most of these tools are designed to target a given set of features of interest, usually spanning a few orders of magnitude in scale, but no single tool can represent the multi-scale anatomy of the reservoir.
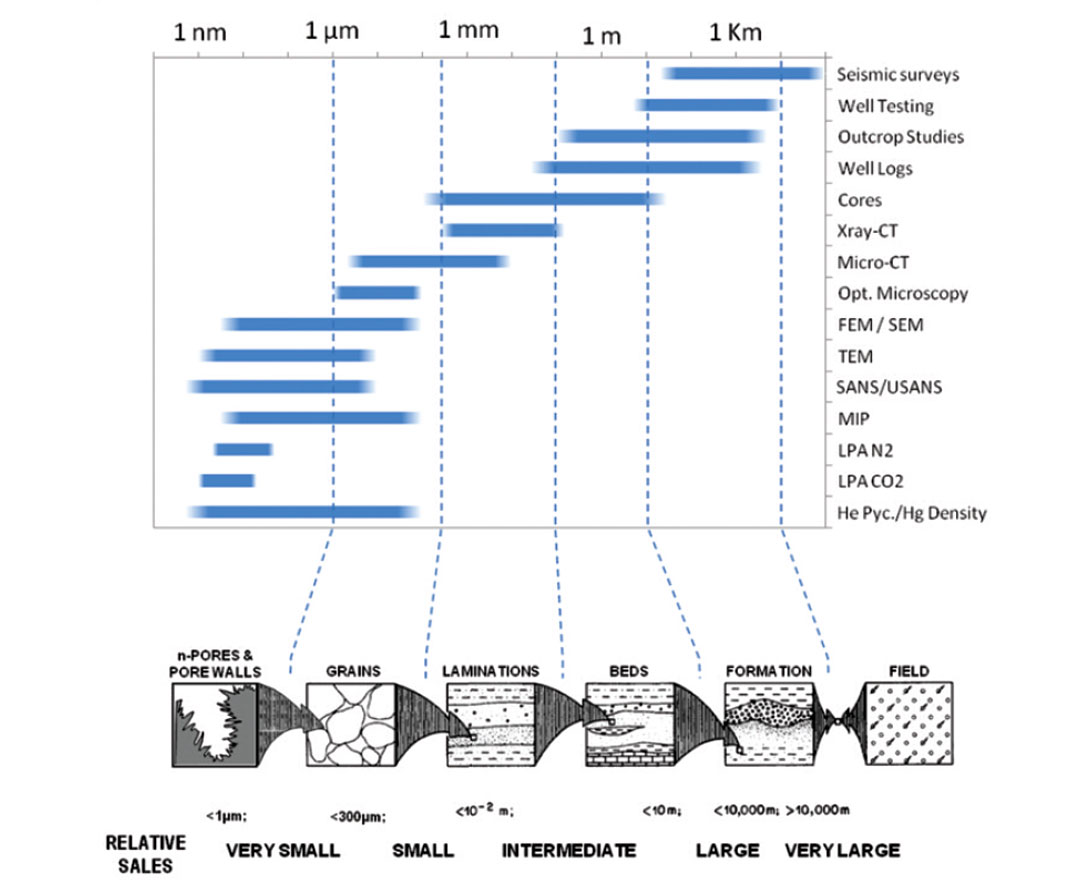
As a general rule, several methods are required in the characterization process allowing for an overlap in the values of the investigated properties. For the purposes of low-permeability hydrocarbon reservoirs, the well spacing area adopted to properly drain a given reservoir volume is reduced. This well-spacing is in most cases one order of magnitude smaller than the short dimension of individual geobodies containing the reservoir lithofacies. As a consequence, the characterization of the near-wellbore (i.e., few 100’s m) and finer scales is most important and technically demanding.
Macroscopic heterogeneities: lithofacies and microlithofacies
The geological characterization of formations typically is ascertained with available outcrops and subsurface core samples. While outcrops provide a better picture of the bedding geometries and stratigraphic architecture, cores better represent the actual reservoir system including diagenesis-related changes and the interaction of native and introduced fluids with the pore system. For the case of halo-oil plays, reservoir lithofacies usually correspond to a few rock types that have marginal reservoir properties and are heterogeneous (Figure 2). The architecture of these small-scale heterogeneities can be inferred from core description because sedimentary rocks are initially layered. However layering may be disturbed and reorganized with varying intensity by organisms. Where this modification is intense the information gathered from the outer face of the cores has a two-dimensional character because the 3-dimensional character of this reorganization is difficult to predict.
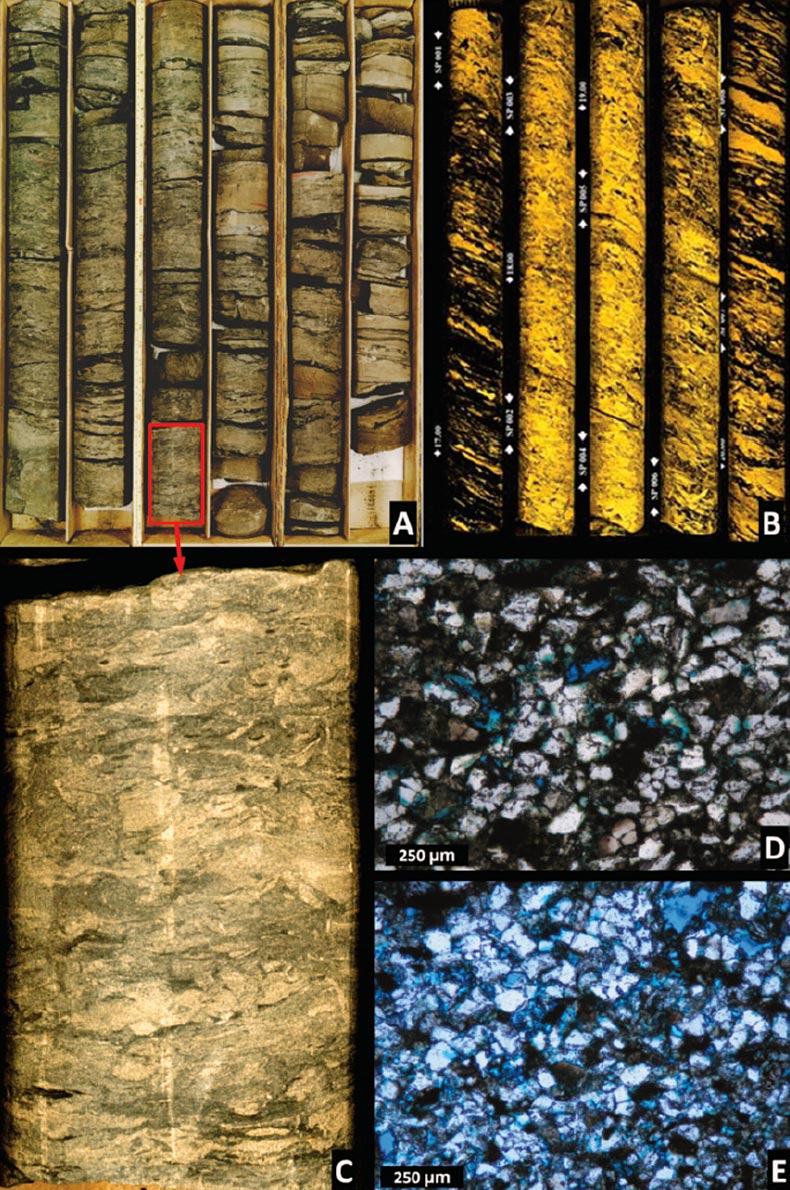
A volumetric characterization of these cm-scale heterogeneities is better achieved using X-ray computed tomography (XRCT), as it can be used to investigate full-diameter cores as well as outcrop samples. This technique relies on the contrast in x-ray signal attenuation coefficients for different lithologies/ compositions as the x-ray passes through the sample. The resolution of the processed volumetric reconstruction extends down to the sub-mm scale, which most of the time allows for the detailed representation of both physicaland biogenic-related sedimentary heterogeneities (i.e., Gingras et al. 2004, Meyer & Krause, 2006; Solano et al. 2012). An example of this type of analysis is shown in Figure 3 for a low-permeability core sample from the Cardium Fm.
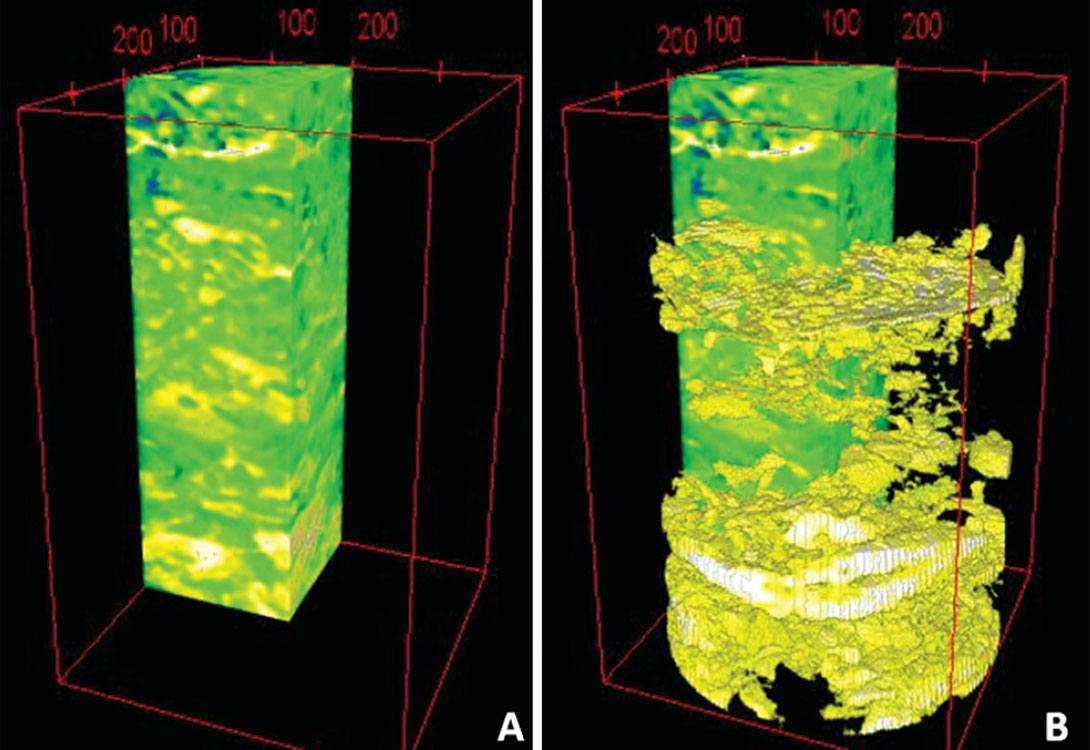
An extension of the XRCT resolution down to a few m-scale is possible using micro-computed tomography ( CT) technology. The complementary application of these two methods allows for the morphological characterization of features of interest with sizes spanning over 5 orders of magnitude.
Porosity and permeability
Characterization of porosity and permeability is of fundamental importance for the proper evaluation of hydrocarbon reservoirs. At the microscopic scale, porosity and permeability are highly dependent on the geometry of the pores and pore throats within volumetrically finite homogeneous systems. These microscopic, locally homogeneous domains are usually found as layered sediments and/or clusters with mm- to dm-scale dimensions imprinting different degrees of heterogeneity to the encasing reservoir. It is then advisable to measure the property variations of subsequently smaller elements in the system (i.e., lithofacies, beds/layers, and microlithofacies).
Porosity of rock samples is traditionally calculated from Helium Pycnometer measurements, from which an accurate value of grain density is obtained using Boyle’s Law expansion as the main principle. The relatively small size of Helium molecules (~0.2nm) ensures that even sub-nm sized pores and pore throats are probed. In addition, the low sorptive capacity of these molecules reduces the errors that might be introduced due to the absorption processes during the measurements. Cui et al. (2009) noted however that helium may be accessible to finer pores than reservoir gases in shales thereby overestimating accessible porosity.
Total porosity values can also be obtained from XRCT datasets. This requires knowledge of the grain density for the investigated rock types expected to be present as part of the analyzed rock volumes. From this, porosity can be calculated on each voxel of the CT volume using the density porosity equation {[ = (m – b)/(m – f ], in which = porosity (fraction); m = matrix density; b = bulk density; and f = fluid density}.
Permeability values from these tight rocks usually range from the sub-microdarcy (<10-3 md) up to the ten’s of md. Permeability is usually measured either with core plugs or full-diameter core samples using unconfined, unsteady-state techniques. For the measurements of ultra-low permeability samples, crushed rock pressuredecay techniques are used instead.
In order to account for the presence of sub-cm scale heterogeneities in these rocks, a pressure decay profile permeameter (PDPK) can be used on slabbed core surfaces. Among several advantages associated with this tool are the relatively small volume of investigation, the upper and lower measurement limits (0.001 – 30,000 md), and the possibility of measuring multiple points in a time-efficient manner. Additional measurements are required using a method that allows application of confining pressure (i.e., pulse decay apparatus) in order to correct the permeability values gathered at ambient pressure to that present at underground conditions; the variations observed by comparing results from different stress conditions usually range between 1 and 2 orders of magnitude (Figure 4).
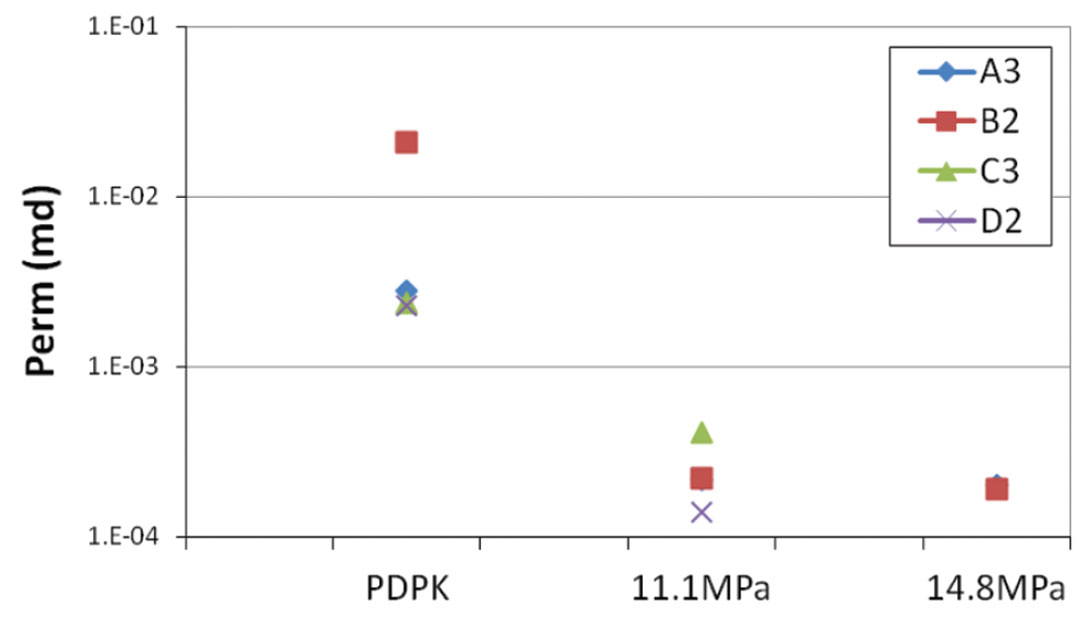
Pore structure
Rocks from typical halo oil plays result from the combination of particles deposited at the time of sedimentation, which often are larger, to particles that grow in diagenetic realms and have a limited size spectrum. Particle size it tight oil plays usually ranges from fine to very fine grained sandstone down to the clay and colloidal size, with small amounts of detrital organic matter material. After consolidation and diagenesis of these sediments, this argillaceoussandstone/ sandy-shale mixture is expected to exhibit pore sizes ranging from the micropore (<2 nm) to the macropore (>50 nm), with a modal peak usually controlled by the amount of clay-sized particles in the porous system. The combination of this wide range of pore sizes and mineralogical variations on the pore walls and pore bodies might locally affect wettability, further complicating multi-phase flow modeling. In addition, multiple equations might be required for the proper modeling of fluid flow through subsequently smaller pores and pore throats.
Several techniques are available for the determination of pore size distributions (PSD) in porous media. Low-pressure adsorption (LPA) and mercury intrusion are among the most widely used methods, together they yield a spectrum of investigation as illustrated in Figure 1. This investigation spectrum is enough to cover most hydrocarbon-bearing rock types. Small angle scattering (SAS) of neutrons and x-rays have also been used recently for the quantification of PSD and surface area in rock samples from
conventional and unconventional reservoirs (i.g., Radlinski et al. 2004, and Clarkson et al. 2013). Besides being a non-destructive technique, a clear advantage of SAS techniques over LPA and MIP is the possibility to investigate PSDs and their variation using relevant fluids (i.e., brine, oil, CO2, CH4) at reservoir conditions. In addition, open vs. non-accessible porosity can be quantified with this technique (Melnichenko et al. 2012). An example of SAS, LPA, and MIP datasets collected from a low-permeability, argillaceous, very fine-grained sandstone sample is shown in Figure 5.
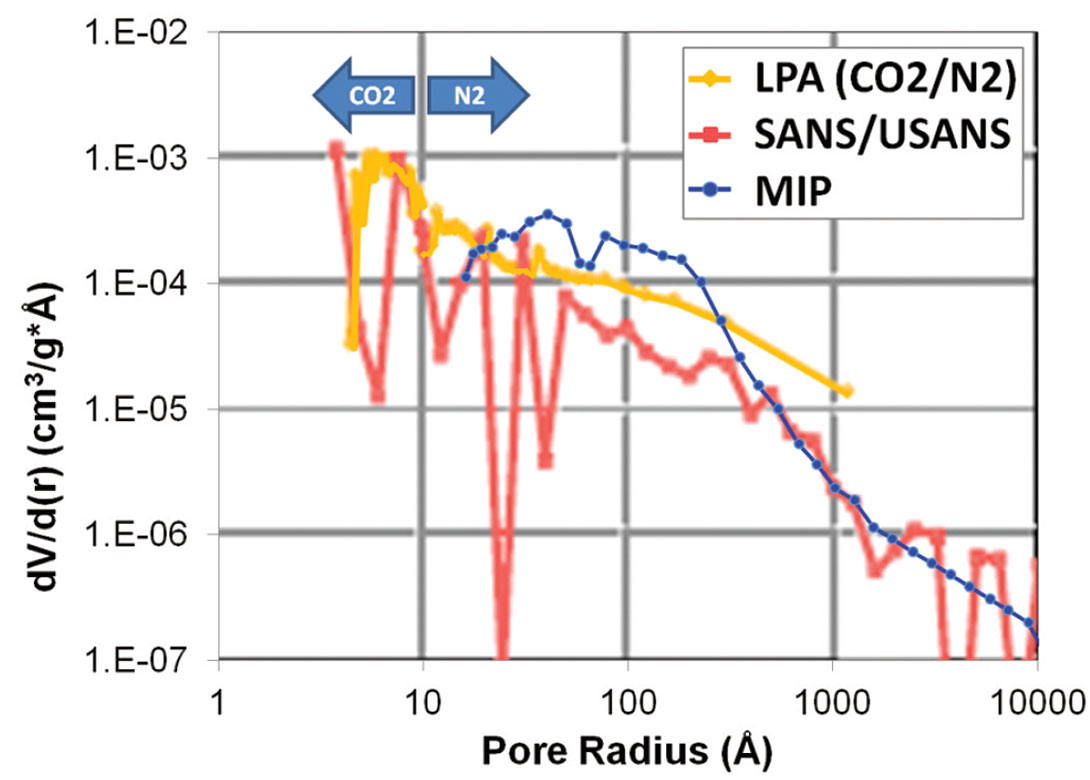
The PSD can be extended to the upper macropores region using complementary, more conventional techniques like SEM and FEM (Figure 6), optical microscopy (Figure 2-D and -E) and CT; an example for the latter is provided in the images and plot in (Figure 7).
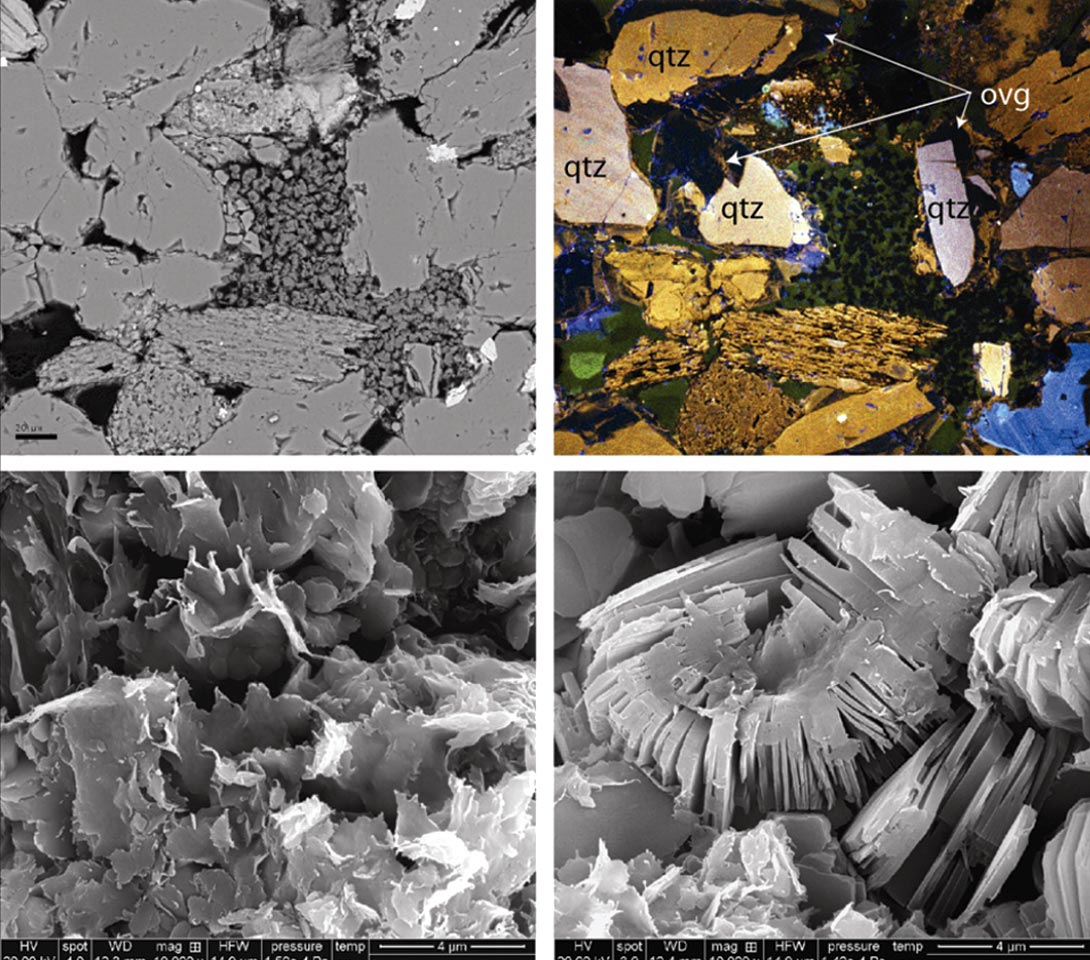
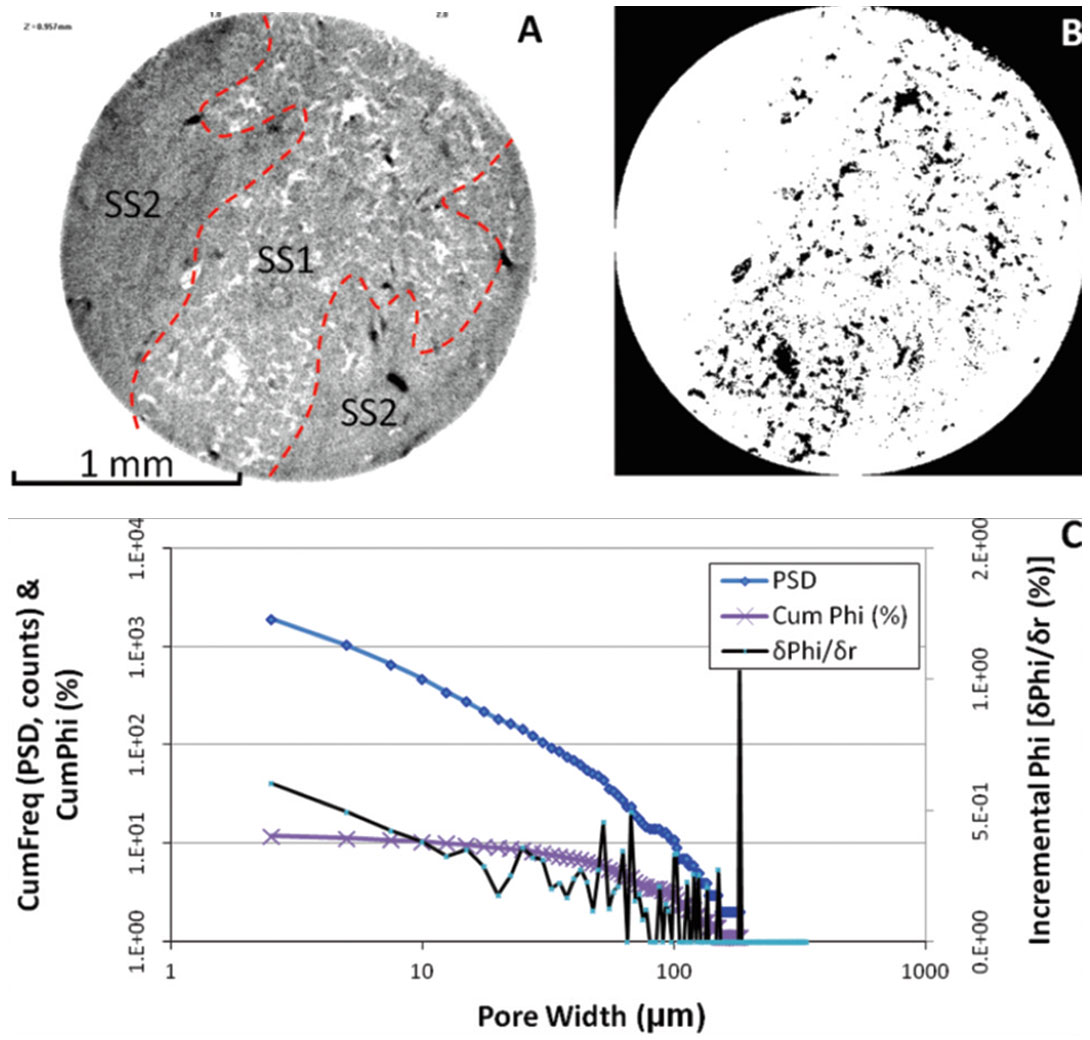
Diagenesis-related variations in the pore structure can be investigated using an SEM/FEM equipped with a combination of different detectors (i.e., SE, BSE, EDX, CL, etc.). In addition, changes associated with native and completion fluids can also be visualized using an environmental and variable pressure SEM/FEM chamber. This capability also provides the opportunity to investigate preferential wettability-related reservoir parameters on grain and mineral surfaces at the pore-scale.
More advanced techniques for surface characterization, such as X-ray Photoelectron Spectroscopy (XPS) and Auger Electron Spectroscopy (AES), are also available; they offer non-destructive chemical compositional analysis and chemical variations in mineralogical and organic grains. XPS yields very accurate and rapid results such as oxidation states and chemical bonds (Figure 8) (Kelemen et al. 2007, Tong et al. 2011). AES enables semi-quantitative elemental composition analysis (down to the 0.1 atomic %), low resolution SEM, elemental mapping, and also depth profiling. Depth profiling in these tools is performed by using an Ar+ ion gun which can sputter and therefore remove layers of 3-25 nm thick from the surface of the sample. All of these combined techniques together provide a more detailed study of the chemical composition variations present at the surface of the samples. This in turn is very useful for investigating controls on wettability, sorption, and other key reservoir properties.
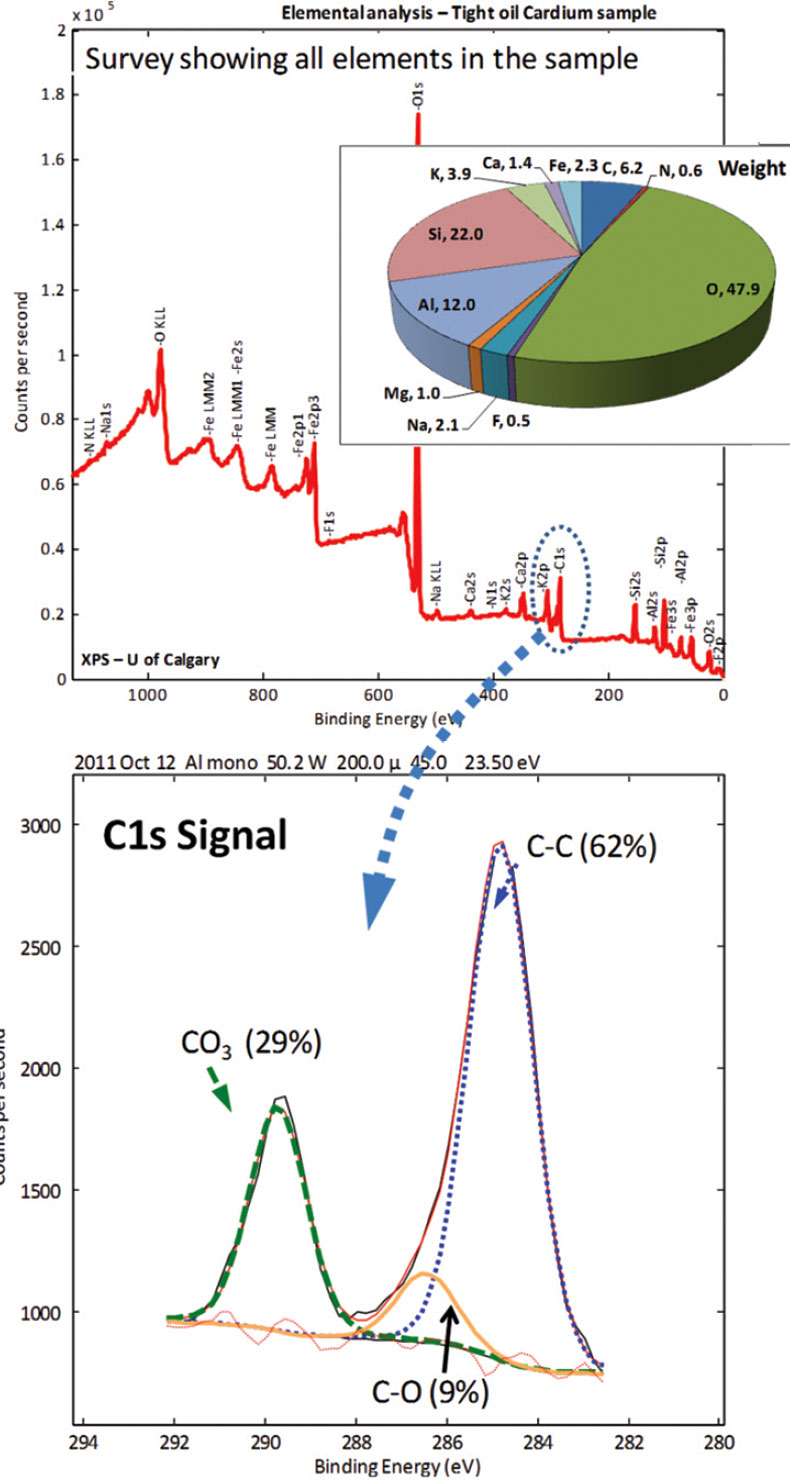
Conclusions
Low-permeability oil reservoirs exhibit a high degree of bodily heterogeneity encompassing different scales within the hosting geological formation. Local variations of porosity, permeability, and pore geometry are variably affected by the compositional nature of the sediments and the depositional environment in which they formed, as well as the evolving diagenetic and tectonic history of the reservoir rocks.
Several characterization tools/ methods targeting these multi-scale heterogeneities are briefly discussed in this work. An important step forward in the characterization process is the investigation of possible correlations between these high resolution datasets with available geophysical and geochemical logs, as well as any regional trends present within the study area. These relationships might work as useful geological proxies for the mapping of the most prospective areas/intervals within these highly complex tight-oil reservoirs.
Acknowledgements
Many of the tools/techniques described above are currently being used in the characterization of unconventional reservoirs as part of the Tight Oil Consortium and IFFAEM in the Department of Geoscience at the University of Calgary. We appreciate the contributions provided to these projects by ARC Resources Ltd., Petrobakken Energy Ltd., TIPM, ORNL, and NIST.
Join the Conversation
Interested in starting, or contributing to a conversation about an article or issue of the RECORDER? Join our CSEG LinkedIn Group.
Share This Article